CONCLUSIONS
From this study of the tektite problem, a number of conclusions can be drawn; clearly they are of widely varying certainty.
The conclusions fall under three main headings: (1) the origin of tektites, (2) the structure of the source volcano on the moon,
and (3) the moon's origin.
ORIGIN OF TEKTITES
We proceed from the conclusion of Chapter 9, that tektites cannot be terrestrial in origin. Then they are probably a kind of lunar obsidian; that is, they are volcanic ejecta, of geologically recent epochs, from one or a number of lunar volcanoes. The steps in the argument are as follows:
Tektites as volcanic rocks
It seems very likely that tektites are the result of volcanic processes rather than of melting by impact. Of the arguments given in Chapter 9 against origin by meteorite (or comet) impact on the earth, a number are also applicable to impact on the moon.
In particular, it is now clear that sites on the moon having the proper chemical composition for tektites are quite rare; tektites are chemically different both from the rocks of the lunar maria and from those of the uplands. This eliminates an impact origin from the moon, though it does not eliminate a lunar origin.
Of the glass-making problems mentioned in Chapter 9, it is true for the moon as for the earth that tektites cannot be made by solid-state transformations nor by evaporation and condensation. The problem of homogenization in the liquid state is just as serious on the moon as on the earth; it implies that the formation of tektite glass must have required times of heating much longer than those involved in impact.
The problem of launching tektites from the moon by impact is not quite as insoluble as launching from the earth; the required velocity is only 2.5 km/sec instead of 6. Nevertheless, as noted in Chapter 9, it appears that impact does not get macroscopic particles up to this velocity.
It seems to follow that tektites must be volcanic rather than impact glasses, wherever they come from.
Tektites as lunar volcanics rather than terrestrial
As noted in Chapter 6, tektites are systematically different from terrestrial volcanic rocks; they are dryer, lower in ferric/ferrous ratio, and contain less volatile elements. These differences are all in the direction of lunar materials. They have been considered to exclude terrestrial volcanics as the source of tektites since the 1920s.
In addition, origin from terrestrial volcanoes is subject to some of the objections brought forward in Chapter 9 against terrestrial impact. There is no reason to believe that terrestrial volcanoes can furnish sufficient velocities; the limiting velocity (Oswatitsch, 1956) for steam at magmatic temperatures (around 1200° C) is about 2.4 km/sec, while 6 km/sec is needed. The upward passage through the atmosphere is impossible unless the atmosphere is removed; the pattern of the strewn field does not fit; and the ablation evidence indicates velocities inconsistent with origin from any terrestrial source, especially one that can furnish maximum velocities around 2.4 km/sec.
We are thus led to the conclusion that tektites are the ejecta of lunar volcanoes. In a way, this conclusion is a very natural one: most of the early students of tektites thought they were obsidians. Charles Darwin (1844), for instance, thought his australite was a volcanic bomb. Likewise all Australian workers up to 1898 referred to australites as obsidian bombs; Dunn (1935) held out for this idea for 40 years. Van Dijk (1879), the discoverer of the billitonites, called them obsidian; Josef Mayer (quoted in Suess, 1900) remarked that moldavites appeared to be a sort of glassy lava; and A. Breithaupt, in 1823 (quoted in Suess, 1900), specifically referred to moldavites as obsidians. The realization that all of these three peculiar obsidians should be classed together was due to Verbeek (1897a,b); he was also the first, in these papers, to suggest that they were the ejecta from lunar volcanoes. Where tektites and obsidians occur side by side, as in the Philippines, there is serious danger of confusion, fortunately cleared up by Koomans (1938). Similarly La Paz (1948) showed that the valverdites were not tektites but weathered obsidian pebbles; and Heide (1936a) showed that the macusanites were not tektites but a kind of volcanic glass.
From the modern standpoint, one immediate objection to the hypothesis of significant contemporary lunar volcanism is the fact that, despite a comparable rate of heat generation, the moon's seismic activity is about 10 orders of magnitude below that of the earth. No volcanic eruption of any size can have occurred anywhere on the moon since the first seismometer was established there in 1969. Many workers feel that the moon has been devoid of volcanic activity since the flooding of the mare basins at -3 to -4 billion years.
But gases are accumulating in the moon as a result of radioactive disintegration. If we assume that the present abundance of potassium in the moon is about 1000 ppm, then the accumulated production of radiogenic 40Ar since -3 billion years amounts to approximately 1/2 ton per square meter of the lunar surface. Of this, according to Hodges et al. (1973), only about 0.4% is escaping by steady flow. Since the moon is not balancing its gas budget on a day-to-day basis, the potential for colossal volcanic eruptions exists even if all volatiles were lost at -3 billion years. If we keep in mind the fact that below 1000 km, the lunar interior seems to be at least in part molten (Nakamura et al., 1973), then there is, at least on the face of it, a case for contemporary lunar volcanism. It must be sporadic, or it would show on the seismograms. The idea of such activity is more plausible, in some ways, than the idea that the ratio of seismic activity to heat flow on the moon is truly 10-10 that of the earth, even over the long run.
At least some recent volcanism appears to be indicated by the morphology of the lunar surface. Within the lunar crater Alphonsus are found a few craters such as that shown in Plate 21 at the junctions of rilles, often surrounded by a halo of black material. The craters are sometimes irregular, and sometimes block the rilles with their debris. Such craters are probably endogenous, and are apparently recent, compared with most lunar features.
THE SOURCE VOLCANO
In this section, we shall assume that a single volcano is the source of tektites. It may in fact be true that there are several, but the number is certainly quite small. We shall try to estimate its period of activity, location, total output, gases, ejection velocity, morphology, source depth, and behavior.
It may seem pointless to try to give a description of a volcano whose mere existence is highly controversial. But by working out its characteristics, we test the idea to see whether it is self-contradictory. We also provide answers to critics who say that a lunar volcano could not function as proposed. We stimulate the observers to test these ideas.
Finally, if the tektites are lunar, then the fact is not at all "terminal", in Urey's phrase; i.e., it has implications for all our ideas about the moon, and even the early earth. The usefulness of the study of tektites is best demonstrated by working out the consequences of the results which have been reached.
Period of activity
The simplest explanation of the radioactive dates noted in Chapter 7 is that the K-Ar, Rb-Sr, and U-Th-Pb dates for a given strewn field all refer to the same event. Then the dates of the strewn fields correspond to the periods of activity at the corresponding vents.
In the case of the Australasian strewn field, it is possible that the eruption which occurred at -0.75 million years carried with it some products of earlier eruptions. This might explain the high-sodium tektites with their -4-million year dates.
It would also help to understand the Muong Nong tektites if there had been several eruptions within a short period of time. The Muong Nong tektites have some fundamental resemblances to terrestrial welded tuffs. This would imply that there had been earlier eruptions at the same site, which had deposited hot microtektites near the vent. The more acid microtektites welded, under the pressure of overlying deposits, to form the Muong Nong-layered tektites. These were then torn loose in a subsequent eruption, perhaps only a few thousand years later, and sent to the earth along with the rest of the Australasian tektites.
So far, tektites are known only from the latter part of the Cenozoic. It would be possible for a very dry glass such as a tektite to resist devitrification for billions of years, as is seen on the moon. Hence the failure to find earlier tektites is puzzling, especially since the larger strewn fields have been repeatedly rediscovered (e.g. the Australasian strewn field, which was independently discovered in Australia, Billiton, the Philippines, South China, Viet Nam, Java, Borneo, the South China Sea, and as microtektites in deep-sea cores; the North American strewn field discovered in Texas, Georgia, Massachusetts, Cuba, and the deep-sea cores; the Ivory Coast strewn field on land and in the cores; and the moldavites in Bohemia and Moravia). Very few discoveries of tektites have resulted from conscious searches; almost everywhere, discovery has amounted to the recognition that something already found is a tektite.
These facts suggest that our list of tektite strewn fields may be reasonably complete. If so, the source volcano did not become active until the latter part of the Cenozoic.
Location
The moon's orbital velocity averages about 1.02 km/sec. If a stone is thrown from the moon with just enough speed to escape the moon's attraction, the stone will still possess this orbital velocity, and will go into a nearly circular orbit around the earth, like the orbit of the moon; then it can never come near the earth. To hit the earth, it must have, in addition to its escape velocity, a component of motion with respect to the moon which will cancel the moon's orbital velocity. Hence the source crater is probably on the moon's eastern hemisphere (which is the side opposite the direction of the moon's orbital motion).
Shute (1966) has made an important simplification of the problem of moon-earth trajectories. She notes that objects
leaving the moon are moving in hyperbolic orbits with respect to the moon's center of mass. At large distances from the
moon, the orbits approach their asymptotes. To a certain approximation, the asymptotes can be replaced by straight lines
emerging from the center of the moon; the error in direction approaches zero asymptotically, and the error in lateral displacement
is generally of the order of the moon's radius, i.e. a few thousand kilometers. The lines from the center can be described
in terms of their intersections with a sphere. If the sphere is taken at a distance of, say, 10 lunar radii from the center,
then the intersection of the actual orbit of the object with this sphere will be not far from the intersection of the radial line
with the sphere. It follows that we can substitute the latitude and longitude of the intersection of the orbit of the particle
with this sphere -- the Shute sphere -- plus the scalar velocity at this point for the full, five-parameter description of the orbit
(the latitude and longitude of the initial crater, and the three components of velocity at the initial point).
For any given initial velocity, it is possible to draw a region on the Shute sphere, from which region a particle must come if it is to strike the earth. For low velocities, the area (see Fig. 43) centers near 0° latitude and 90° E longitude, exactly opposite the direction of the moon's orbital motion. For higher velocities, we are dealing with orbits whose apogees are beyond the moon. Hence two areas on the Shute sphere are involved, one on the front side, for direct orbits to the earth, and the other on the far side, for movement out to the apogee and return to the earth. For ejection velocities greater than 3.1 km/sec the apogee ceases to exist for orbits to the earth (the orbits are hyperbolic with respect to the earth) and hence the spots on the Shute sphere are real only on the near side. The spot approaches the center of the moon's visible face for very large velocities.
In the study of Chapman (1964), it was found that the velocity vectors for orbits to the earth all point in the same direction, namely toward the lunar equator and 90° E. This fact is illustrated in Fig. 44, which D.R. Chapman kindly furnished. The initial craters are distributed widely over the moon's front face, because Chapman was interested in impact phenomena; for these, he believed that the most favorable angle to the lunar surface for the formation of tektites was around 30° to 60° to the horizontal.
If tektites come from lunar volcanoes, however, their ejecta should be nearly vertical; then the crater on the lunar surface should be just about directly below the point on the Shute sphere. It follows that the location of the source crater should be toward the center of the eastern hemisphere, and not far from the equator.
The remote-sensing data summarized in the frontispiece of Gose (1973) indicate that the area involved has not yet been overflown; it should show a simultaneous low of Al/Si, enhancement of gamma-radioactivity, and enhancement of the alpha-particle flux. Some trends, especially in K/U (Metzger et al., 1973) and in the rare earth ratios (S.R. Taylor et al., 1972), seem to indicate that these ratios reach more favorable values as one goes eastward toward 90° E.
The absence of inclusions of typical lunar basalts in tektites suggests that the vent is surrounded by deposits of considerable depth.
Total output
The majority of the known tektite mass is in the North American strewn field; it amounts to 1 billion tons, or about 0.4 km3
Volcanic gases
The principal gas in terrestrial volcanism is steam. This cannot be the case for lunar volcanoes; if water is passed over a lunar magma at a temperature of 1200° C or so, it will be largely reduced to hydrogen. Consider the reaction:

The ratios of the partial pressures of the gases obey the law:
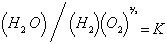
where K is the equilibrium constant. From the JANAF tables (Stull and Prophet, 1971) at 1500° K, log10K=5.725 if pressures are in atmospheres, or 3.225 if pressures are in N/m2. The partial pressure of oxygen in equilibrium with a lunar magma at this temperature is about 10-13 atmospheres or 10-8 N/m2; from Walter and Doan (1969) we have for tektites, at 1500° K, the result that log10PO2 is -12.61 atmospheres, or -7.61 N/m2. From the latter figure, the ratio, H2O/H2 is 0.26 The mean molecular weight is 5.30; the mean specific heat at constant pressure, Cp, is 2.85.
This mix, of four parts hydrogen to one part steam, is probably the characteristic lunar volcanic gas.
The limiting velocity Vlim for gases at absolute temperature T escaping from an enclosure into a vacuum is:

which works out at 6.0 km/sec for 1500° K and our mixture of hydrogen and steam.
By contrast, for water at this temperature, Vlim=2.4 km/sec; this is evidently an important advantage which lunar volcanoes have for launching particles into ballistic trajectories.
It might also be possible in a lunar volcanic explosion to shock a portion of the ejecta. The mere release of contained gases could not produce shock because the required pressures of 1010 N/m2 (100 kbars) or the like could not be contained by the lunar crust (N.M. Short, personal communication). However, very violent chemical reactions can produce shock, and thus might conceivably account for the coesite found by Walter (1965).
Morphology
The ballistic effects of volcanic gases on the rocks around a crater constitute a problem in hydrodynamics in which the viscosity of the fluid is unimportant. The restraining force on the material is largely gravity. For this case, the relevant non-dimensional number is the Froude number, Fr, which measures the ratio of inertial forces to gravity:

Here V is the gas velocity, g the acceleration of gravity, and l a typical dimension (e.g. the size of the rock). For the same rock size, the lunar Froude number in a volcanic eruption will be 50 times larger than in the terrestrial case, because of the increased gas velocity and the decreased gravity. It follows that we need not expect a lunar volcano to look like a terrestrial volcano of the same size. It should be much more explosive; and the absence of an atmosphere should contribute to the violence.
Since the source volcano must be among the most recent lunar features, and since it is well known that the ray craters are also among the most recent lunar craters, it is natural to ask whether the tektite source is a ray crater.
It is usual to think of ray craters as the prototypes of impact craters. The original argument, however, which led us to this conclusion is obsolete; it regarded the rays as light-colored rock flour thrown out of the impact crater: "powdered rock is almost always lighter than the original solid" (Baldwin, 1949, p. 163). We now know that lunar fines are darker, not brighter, than the lunar rock (Shoemaker et al., 1968, p. 4028). Ray craters give strong radar reflections and strong thermal anomalies, which means that rock is exposed.
If rays are regions where exceptional amounts of rock are exposed, then we cannot comfortably say that the rock was thrown from the central crater, because, as we noted above, most of the material which is sent long distances on the lunar surface is fine material.
It is more logical to think of the rays as paths of gaseous blasts issuing from time to time from the central crater. These would blow the accumulated dust off the tops of the rocks; in this way we can understand how the rays can appear to be the loci of small ray craters (Oberbeck, 1971). The small ray craters, with their haloes of blocks, exist everywhere, but in most places the rocks have been covered by dust; only along the rays from the great lunar craters is the dust removed. For this reason, the long-wave radar does not see the great rays (Thompson, 1970); even in the non-ray areas it looks right through the dust, and hence sees no more blocks on the great rays than elsewhere.
Since the rate of growth of the dust layer is about 0.4 g/cm2 per 1 million years, it follows that the gaseous blasts must occur much more frequently than crater-forming events, volcanic or not, in order to maintain dust-free surfaces.
It thus does not appear unreasonable to suppose that the tektite source crater is a lunar ray crater.
Source region of the magmas
The lunar exterior differs from tektites in being more completely outgassed. The difference in the lead isotopes is particularly striking: the 206Pb/204Pb ratio in tektites is about 18, while in the outer regions of the moon, the Apollo and Luna missions have usually found values near 200. The explanation is that 204Pb is primordial; it must have been lost from the outer part of the moon in an early heating episode. On the other hand, 206Pb is a decay product of 238U, which is very refractory. Accordingly, tektites are not derivable from typical lunar surface rocks.
On the other hand, in the Apollo 17 orange glass, Tatsumoto et al. (1973) and Silver (1974) found evidence of a lead much nearer a terrestrial lead; and in fact Silver remarks that this lead appeared to have separated from an assemblage of U-Th-Pb much like that believed to exist in the earth's mantle.
The tektite source region is probably at some depth. Below 1000 km, the lunar interior is at least partly molten (Nakamura et al., 1973). Moreover, the magnesium-rich bottle-green microtektites may be the lower part of a differentiation column, that is, portions of the sub-crustal material of the moon carried up by the eruption.
It is even imaginable that the nickel-iron spherules of tektites, which are always found embedded in low-calcium high-aluminum tektites, are of internal rather than external origin. Conceivably they are somehow related to the core of heavy elements found by Nakamura et al. (1974).
Electromagnetic behavior
The presence of magnetism in Muong Nong tektites must mean that the moon now possesses the power to magnetize rock. The most plausible explanation is lightning (Čap, 1972). This is a normal accompaniment of ash flows. It would connect the magnetism in Muong Nong tektites with the magnetism in lunar rocks, which is typically strongest in lunar breccias. The view that the lunar breccias are the product of ash flows whether induced by impact or by volcanism has been brought forth by numerous authors, cited in Pai et al. (1972).
The lightning furnishes a possible explanation of the orange spots sometimes seen on the moon (Middlehurst, 1966). The spectrum of the orange spots at Aristarchus was reported by Kozyrev (1963) to be that of H2. The color of the H2 spectrum agrees with that reported for the spots. The transitions which give rise to the color are between levels which are ten volts or more above ground; it follows that the required temperatures for thermal excitation would be in the tens of thousands of degrees. Only an electrical method of excitation is plausible (Mills, 1970).
THE ORIGIN OF THE MOON
The lunar origin of tektites strongly suggests that the moon must have formed by fission of the earth. Only in this way is it possible to explain the remarkable geochemical resemblance of tektites to terrestrial materials. The resemblance is so strong that at the present moment a large number of geochemists insist that tektites must be the direct products of the earth, in defiance of the physical impossibilities outlined in Chapter 9. They have repeatedly drawn attention to features of tektite composition, such as the low nickel content (Taylor, 1962a), or the high degree of differentiation (Barnes, 1958a), or the evidence of terrestrial U-Th-Pb ratios (Taylor, 1973) as evidence of the terrestrial origin of tektites, only to have the argument collapse beneath them as the same features were found on the moon. Even today, many geochemists regard the oxygen isotope ratios, especially on 12013, as decisive proof that tektites are terrestrial rather than lunar. It is not possible to ignore these arguments; tektites are indeed much more like terrestrial rocks than would be expected for some chance assemblage.
It seems to follow that deep inside the moon there must be material very much like the mantle of the earth; in fact it must be more like the mantle of the earth than like the ultrabasic rocks which are the source regions for the lunar surface rocks. This remark was made (O'Keefe, 1972) before the finding of the earth-like lead isotopes in Apollo 17; from this finding, Silver (1974) supports the above statement about earth-like material in the moon's interior.
The very small size of the lunar core is particularly striking in this connection. It constitutes a strong constraint on hypotheses of the origin of the moon; many otherwise satisfactory ideas can be ruled out. It seems to mean either that the moon is of very primitive character, like a carbonaceous chondrite, with most of its iron oxidized, or that it is the product of fission (O'Keefe and Urey, 1975).
The fission hypothesis for the origin of the moon seems to imply a period of intense heating, from the outside, and a loss of most of the moon's original mass (O'Keefe, 1969c). This would explain why the parts of the moon reached by most of the lunar probes show some systematic differences from tektites. The lavas constituting most of the moon's outer surface were erupted early in the moon's history, when the moon's heat was near the surface (see, e.g., Toksöz et al., 1973). At present, source regions for lunar volcanism are necessarily very deep; they are therefore drawing on the material which suffered least during the period of ablation, and which therefore is most similar to unaltered terrestrial mantle materials. This may be why tektites are more like terrestrial rocks in many ways than like the rocks of the lunar surface.
SUMMARY
The available data on tektites cannot be fitted into the hypothesis that tektites are the product of terrestrial meteorite or comet impact. The discrepancies occur in at least three independent fields (cratering, glass-making and aerodynamics) and in each field amount to three or more orders of magnitude.
The only viable alternative seems to be volcanic ejection from the moon. A reasonably consistent picture of these events can be put together. They are probably rare paroxysms from a center or centers somewhere in the moon's eastern hemisphere, starting a hundred kilometers or so below the moon's surface, and involving ash flows and associated lightning.
If we have argued correctly, then the insistence of the geochemists that tektites are terrestrial must mean that the moon has an earth-like center, and was probably produced by fission, followed by the ablative loss of most of the original mass.