THE CHEMICAL COMPOSITION OF TEKTITES
RELIABILITY OF ANALYSES
Barnes (1939) compiled the tektite analyses available up to that time. Of these, the analyses prior to 1930 are not generally useful; many even lack TiO2. Of the analyses made in the 1930s the two bediasite analyses reported by Barnes (1939) are to be rejected; the MgO/CaO ratio in them is highly discordant with all other analyses. The analyses of F. Raoult, reported by Lacroix in various papers of this period, are comparable with good modern wet-chemical analyses.
In most wet-chemical analyses, there is a tendency to overstate the content of water and ferric iron. Friedman (1958) and Gilchrist et al. (1969) showed that water is present at the level of 0.01% (or less); it is thus below the limit of detection by wet-chemical methods. The ferric/ferrous ratio was found to be 0.05 for bediasites by Thorpe et al. (1963); values much over 0.15 are rare in modern analyses.
With respect to the trace elements, the work of Cohen (1959) on lithium and
rubidium has been criticized by Schnetzler and Pinson (1963). The value
of 7700 ppm for barium in moldavites is startling and very important if correct
(Vorob'yev, 1960a); unfortunately accompanying values for barium in
indochinites (Vorob'yev, 1959b) exceed those of other workers by a considerable
factor.
CENTRAL COMPOSITION
Table V gives the chemical composition of a representative tektite, in particular an australite. For most elements, it is taken from S.R. Taylor (1966), australite No. 28, 11947D. This australite is closely similar to the normal australite of Chapman and Scheiber (1969), No. 45, AN 99. Where Taylor does not have data, the gaps have been filled with other australite analyses as shown in the notes, or, in one or two cases of non-critical elements, with other kinds of tektites.
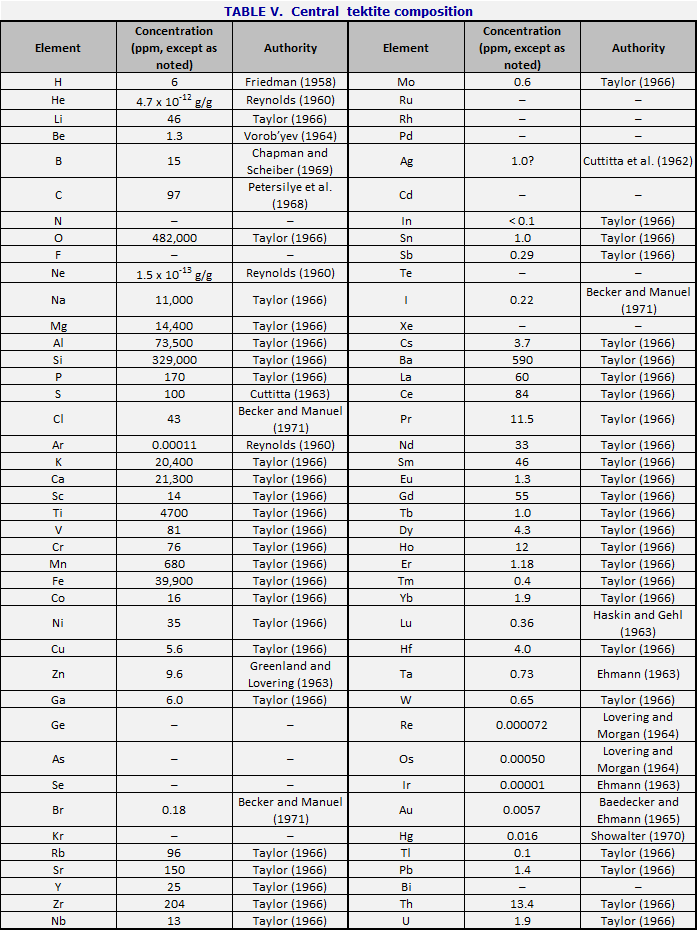
In Figs. 28 and 29, the composition is compared
respectively with that of the U.S.G.S. standard granite, G-1, and the standard
basalt, W-1, using the 1972 recommended values (Flanagan, 1973). (These
rocks are not necessarily representative; they are chemical standards, which
are useful here because they have been fully and carefully analyzed, and
because they give an adequate idea of the two principal rock types. ) The
arrangement is the long form of the periodic table. The ratio of the
element concentration, in grams per gram, to the concentration of the standard,
is represented logarithmically by bars going upward for excesses, and downward
for deficiencies.
It is seen that, particularly on the left side of the periodic table, the
tektite central composition falls between the granite and the basalt; where the
bars are upward in the granite, they are downward in the basalt, and vice
versa. Exceptions are the volatile elements: hydrogen, and a large
group on the right side of a table enclosed by a line. For these
elements, tektites are deficient as compared with both standard rocks.
It is therefore logical to compare with an intermediate rock; Fig. 30 shows the comparison with the standard andesite, AGV-1 (Flanagan, 1973); it is obvious that this is a better match than either the basalt or the granite, but the deficiencies on the right side of the table remain conspicuous.
This result is
surprising, in a way, because the SiO2 content is 70.4% for
the central tektite, and 72.6% for G-1, while for AGV-1 it is 59.0%. We
might thus have expected the tektite to be close to the granite than to the
andesite, because it is well known that the chemical composition of igneous
rocks is largely determined by the silica content. However, it was
pointed out by Mueller (1915) that tektites could be distinguished from
terrestrial igneous rocks because the ratio (FeO + MgO)/Na2O + K2O)
was higher for a given silica content. This ratio decreases with
increasing silica content, so that Mueller was finding the above-mentioned
result, namely that tektite composition resembles (for most elements) that of
terrestrial rocks of lower silica content. (We refer here to the left
side of the periodic table.) Suess (1933) comments similarly; so do
Loewinson-Lessing (1935). Barnes (1939) used this fact as the basis for
his conclusion that tektites are for the most part formed from terrestrial
sedimentary rocks. Urey (1958a, b) commented similarly. The same
idea was expressed by Cherry and his collaborators (Cherry et al., 1960;
Taylor, 1960, 1962c; Cherry and Taylor, 1961; Taylor and Sachs, 1961; Taylor et
al., 1961).
Later, Taylor suggested that sedimentary processes could enhance the silica content in the way required. In particular, Taylor studied the Henbury sandstone (see Fig. 31); here it is clear that sedimentary processes are enhancing the silica content, because some of the sandstone has as much as 94% SiO2 (Taylor and Kolbe, 1965). By proper choice of sandstone, Taylor was able to come close to the australite composition, both in silica content and in other non-volatile elements (Taylor, 1966).
The discrepancy in hydrogen (i.e. water content) was commented on by Suess
(1900, p. 247); in fact, the field test to distinguish a tektite from an
obsidian is to heat it with a blowtorch or blowpipe; the tektite melts with at
most a few bubbles, while the obsidian foams (La Paz, 1948). Terrestrial
igneous rocks tend to have about 5000 ppm water, and sedimentary rocks several
times as much. In an impact, however, the water may escape, at the price
of turning the rock into a mass of bubbles (see e.g. Taylor and Kolbe, 1965).
On the right side of the periodic table, there are major discrepancies in many
of the elements which are volatile at temperatures of the order of 1000°
C. These discrepancies are difficult to measure by the usual methods of
spectrochemical analysis. The elements are scarce; they volatilize at
temperatures below those which are optimum for the metals on the left side of
the diagram; and their spectra often have the important lines in relatively
inaccessible parts of the ultraviolet. Hence these discrepancies tend to
be overlooked. Nevertheless Preuss (1935) and Heide (1936b) noted that
tektites are lower in copper, germanium, tin, and lead than their suggested
terrestrial comparison material (Norwegian loam); similarly Taylor (1966)
remarked on the deficiencies in copper, lead, tin, thallium, iridium, and
bismuth compared with the Henbury impact glasses.
Similar discrepancies are conspicuous when lunar rocks are compared with terrestrial rocks of similar type. In Fig. 32 a basaltic clast, 14321.223 from Apollo 14 (Wänke et al., 1972) is compared with W-1. In Fig. 33 the central tektite composition is compared with lunar sample 12013, the only lunar sample of acidic composition for which substantial trace element data exist. The comparison is unsatisfactory in several respects; but it suggests that the systematic discrepancy in the volatile elements is removed.
There is also a marked difference in the ratio of ferrous to ferric iron, which does not appear on the charts. Terrestrial acid igneous rocks or terrestrial sandstones typically have ferric/ferrous ratios of the order of 1, or even more. For tektites, as mentioned, the ratio is generally less than 0.15.
The chemical parameter which underlies the ferric/ferrous ratio is the oxygen fugacity, which is numerically equal to the equilibrium partial pressure of oxygen, PO2, that is, to the value of the partial pressure which would be in equilibrium with the glass at the given temperature. Walter and Doan (1969) report preliminary values as follows:
log10PO2 = 9.13 - 32,600/T
where PO2is in atmospheres, and T is the Kelvin temperature. The corresponding number in N/m2 is greater by a factor of 105. The relation yields 10-9.6 N/m2 (or 10-14.6 atmosphere) at 1100° C, about six orders of magnitude below terrestrial rocks at this temperature (Walter and Doan's value of 10-17.6atmosphere for 800° C is a slip; it should be about 10-21.3 according to their unpublished charts). More measurements are needed.
Vorob'yev (1959a) found magnetite spherules, often hollow, in surface cavities of Philippine tektites. Kleinman (1967) found magnetite inclusions some of which had nickel-free iron cores, as if the tektites had formed in equilibrium with metallic iron, which later oxidized (verbal suggestion by L.S. Walter). This would again suggest a low oxygen fugacity.
Brett (1967) has noted a relation between the nickel content of metallic spherules in tektites and impact glasses, and the presence or absence of a halo of iron oxide in the surrounding glass. It appears that for impact glasses, a portion of the iron oxidizes and dissolves in the glass, leaving the spherule nickel-rich. For tektites, on the other hand, the nickel enrichment and the iron oxide halo are both missing. This result seems to imply that the oxygen fugacity in tektites is much lower than in impact glasses, so that they do not tend to oxidize the iron.
THE PRINCIPAL FAMILIES OF TEKTITES
When one is confronted with a hand specimen of a tektite, it is usually possible to find out where it has come from by analyzing it. Most tektite analyses are carried out in weight percent of major oxides. By comparison with the central composition (Table V), the North American tektites (see Table VI, cols. 15-19) are systematically low in CaO and MgO. Moldavites tend to have high silica (over 75%) and high K2O (over 2.5%). Ivory Coast tektites usually have low silica (under 70%) and often have Na2O>K20. Although Aouelloul glass is broadly similar to Darwin glass, both being in the range near 85% SiO2, the Aouelloul glass has higher CaO and MgO.When careful studies are made, including microtektites and rare types of macrotektites, it is seen that the range within one strewn field is often much greater than the range from one strewn field to another. In Table VI are given the major element compositions of tektites from various strewn fields. In the major fields, the attempt is made to choose examples which show the range in silica content, and the corresponding changes in the other major elements. Table VII lists the corresponding minor and trace elements, where these have been determined.
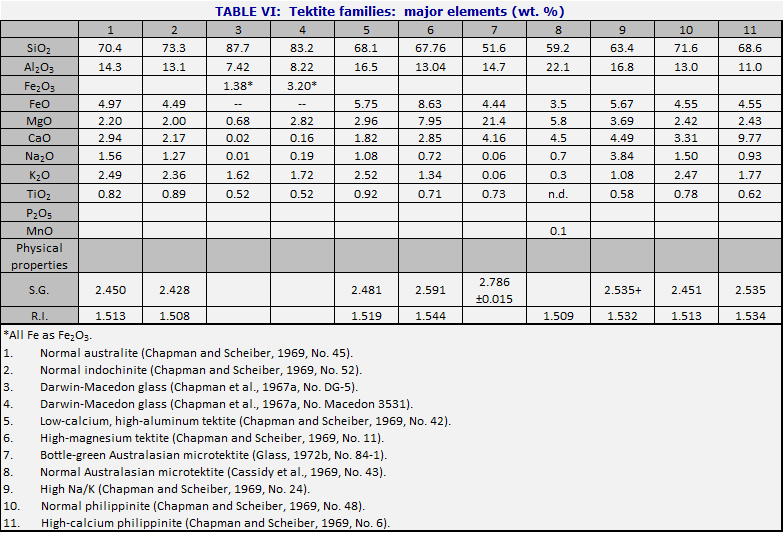
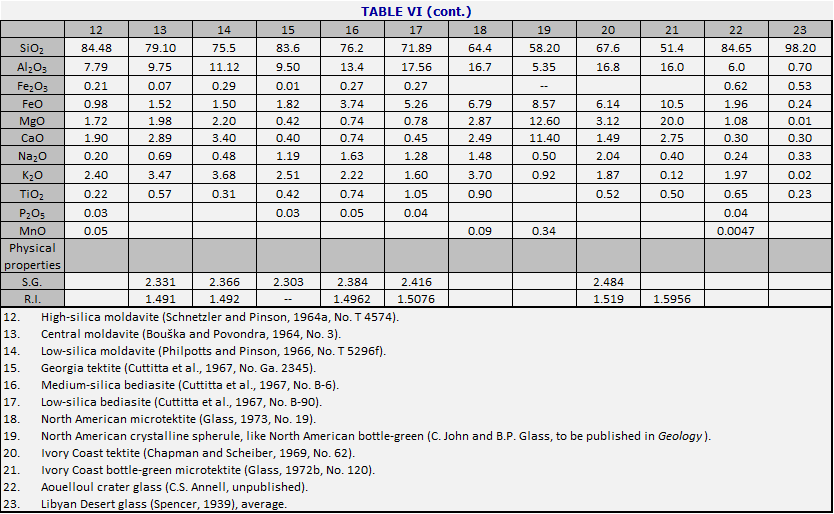
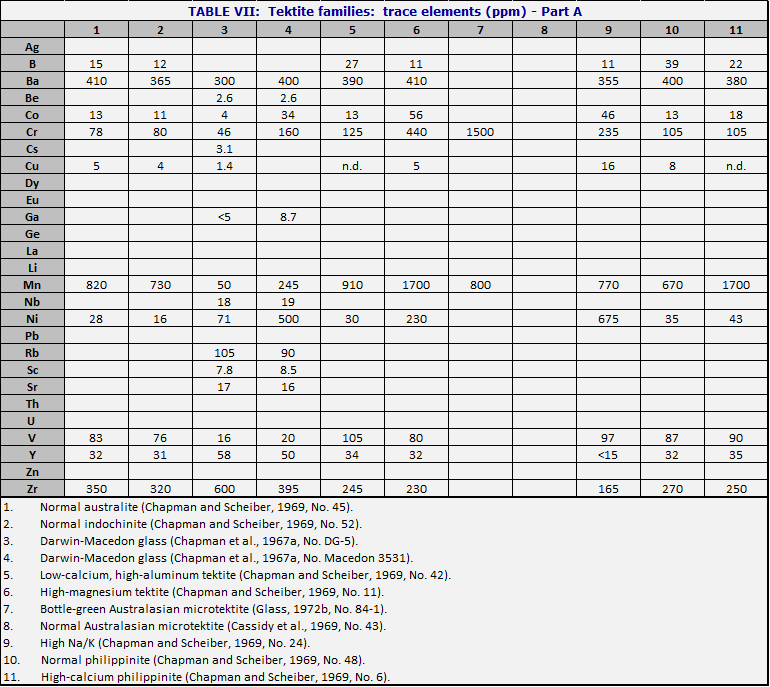
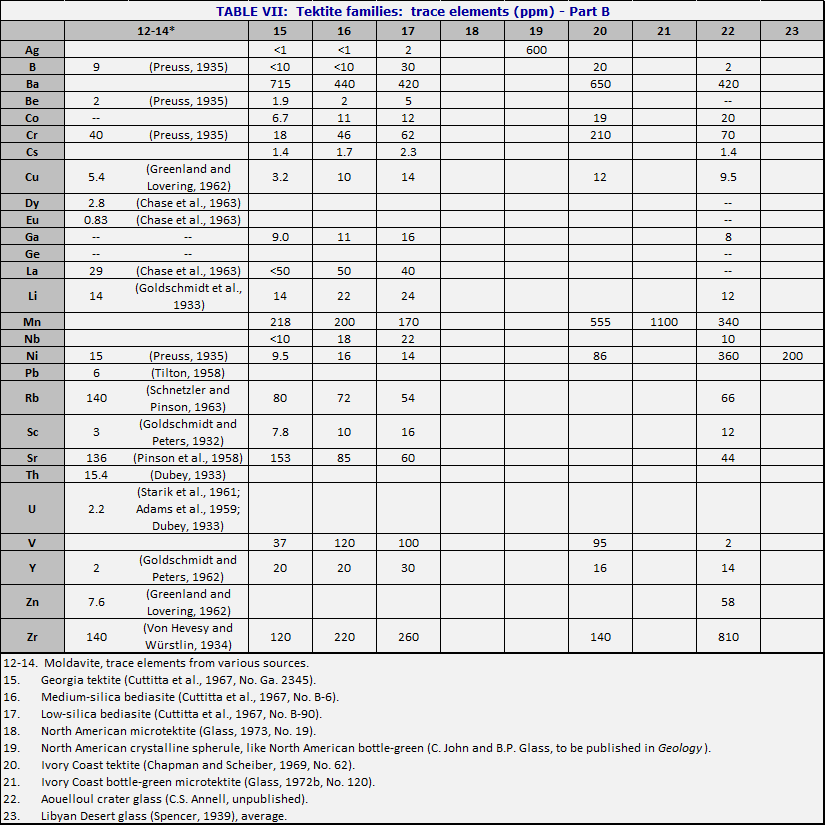
The variety of tektite types is such that some kind of overview is needed. In Figs. 34 and 35, the numbers of the points correspond to numbers of the columns in Tables VI and VII. Fig. 34 diagrams the more important types of tektites within the Australasian strewn field. To construct the figure, the weight percent MgO was taken from the analysis, added to CaO and 0.05 SiO2; call the sum s. Then MgO/s is plotted vertically upward from the base of the triangle, and similarly for the other two directions. The central composition of Table V is that of the normal australite, No. 1, near the middle of Fig. 34. Normal philippinites (10) and normal indochinites (2) are nearby.
The principal variations in tektite composition are toward high magnesium content (bottle-green tektites, 6 and 7), and toward high silica (Darwin glass, 3 and 4). There is also a less common class of high-calcium tektites (11). An important class of microtektites lies on a spur pointing away from the SiO2 vertex (8). The curious class of the low-calcium, high-aluminum tektites (5) is interesting because the nickel-iron spherules (Chao et al., 1962, 1964) occur in tektites of this composition. Even in Aouelloul glass (Chao et al., 1966a; Chapman and Scheiber, 1969), which is so different from most of the philippinites and indochinites where most of these spherules have been found, there is a narrow zone of glass of similar composition (O’Keefe et al., 1971).
The high-Na/K tektites (Chapman and Scheiber, 1969) are of interest because their major element analyses resemble terrestrial andesites (apart from the usual lack of water and ferric iron). They resemble Ivory Coast tektites in composition, and their dating is a major puzzle (Chapter 7).
Fig. 35 represents the other tektite strewn fields just as
Fig. 34 presents the Australasian. Note that the North American tektites lie
near the SiO2 vertex not because they are silica-rich but because
they are poor in CaO and MgO.
TRENDS IN COMPOSITION
Correlations with silica content
The first-order description of chemical trends in tektites is that silica is negatively correlated with other oxides. Variation diagrams (plots of oxide concentration versus silica concentration) were prepared by Suess (1900, p. 236), Summers (1913), Suess (1914), Barnes (1939, figs. 93, 94) and Cassidy (1958). Cherry and Taylor (1959) sought to explain the correlation by assuming a mix of arkosic sandstone with a hypothetical cometary composition resembling the silicate portion of a chondritic meteorite. This idea became untenable (Taylor et al., 1961) when more refined analysis showed that magnesium in tektites is positively correlated with alkalis such as Na2O; it was replaced by the hypothesis that tektites are a mixture of shale plus pure quartz in varying proportions.
Lowman (1962), using Barnes’s (1939) compilation, compared the variance of tektite compositions with those of randomly chosen igneous and sedimentary rocks in the same range of silica content; the tektites were markedly narrower in range, especially by comparison with sedimentary rocks. Cuttitta et al. (1963 a, b) and Chao (1963) also noted the positive correlation of the alkali elements with one another, and the general negative correlation of oxides with SiO2; they found Al2O3 in indochinites to show no correlation. Tatlock (1966) and Meadows et al. (1967) showed that the variances become even narrower when modern analyses are used. As noted above, Taylor and his co-workers later attributed the correlation with silica to sedimentary differentiation (quartz grains enriched relative to weaker materials) rather than to a mixing process in the literal sense.
The K/Rb ratio was studied by Ahrens et al. (1952), Taylor (1960), Pinson et al. (1965), Taylor et al. (1967); values converged toward 175-195. These resemble the ratios in terrestrial rocks, and do not support the hypothesis of Ehmann and Kohman (1958b; Kohman, 1959) that tektites come from outside the solar system.
Similarly Setser and Ehmann (1964) found a value near 30 for Zr/Hf in tektites, as in some sediments. Greenland and Lovering (1965) found a tight positive correlation of MnO with FeO. Ehmann and Showalter (1971) found that sodium is positively correlated with iron in australites, although the correlation is negative in subgraywackes. Rybach and Adams (1969a, b) found that the Th/U ratio is 2.6 for Ivory Coast tektites, but is considerably greater than 4 (the cosmic value) for other tektites. For K/U they found 13,000, similar to terrestrial values.
The question whether these correlations are simply the result of the variation of silica was attacked by Leake (1970) and Walter and Shadid (1970) using Niggli values. Both papers concluded that the compositional trends do not match any of the proposed theories (igneous differentiation, sedimentary differentiation, differential volatilization).
Some light is shed on these relations by the findings of
Glass (1970a) and L. S. Walter (unpublished manuscript kindly communicated) to
the effect that compositional trends among tektites are matched by
compositional trends within individual tektites, as if the tektites were in
some sense, composites of microtektites (see Chapter 4).
Correlations with nickel, chromium, and magnesium
All of the above are more or less explicable in terms of a mixing model having silica as one end-member, and a rock of intermediate acidity as the other. But in some groups of tektites, there is the appearance of a different kind of mixing relation, in which the basic end-member is an ultrabasic rock, conceivably like a bottle-green microtektite, and the acidic end-member is something like a moldavite.
The first evidence for the ultrabasic end-member seems to have been the findings of Preuss (1934) that chromium and nickel are enhanced by a factor 10 in billitonites as compared with australites. He noted that although nickel enhancement occurs in impact glasses, there is no enhancement of chromium as in tektites. He discussed the matter further in his great spectrochemical study (Preuss, 1935); Heide (1936b) also referred to it; later, Heide (1938b) mentioned that the nickel-rich and chromium-rich tektites are distributed along a line from Billiton through Borneo, Cambodia, Cochin-China, with nickel-poor tektites on both sides. Taylor (1962b) and Schnetzler and Pinson (1963) reiterated Preuss’s argument, namely that the simultaneous enhancement of chromium and nickel cannot be due to meteoritic contamination.
Chao (1963) showed that nickel plus chromium is weakly correlated with MgO plus FeO in bediasites, but strongly correlated in australites. Taylor (1964) noted two australites enriched in nickel, chromium and cobalt. Pinson and Griswold (1969) and Ehmann and Showalter (1971) again commented on the Ni-Cr relation.
The subject was illuminated by Chapman and Scheiber (1969)
who identified an important class of tektites in the Australasian strewn field,
namely the high-magnesium tektites, which are enriched in magnesium, nickel,
cobalt, and chromium. As Chapman and Scheiber remark, and as was later shown by
Glass (1972b), the high-magnesium tektites agree in composition with the bottle-green
microtektites in the range from 63 to 75% SiO2. Apparently the
nickel-rich tektites of Heide and Preuss are the same as the high-magnesium
family of Chapman and Scheiber. Unfortunately, owing to the very small amount
of material available, there is no direct demonstration that the bottle-green
microtektites are in fact nickel-rich; that they are chromium-rich was found by
Glass (1972b).
High copper and boron in tektites
Chapman and Scheiber (1969) found that many Muong Nong
tektites are enriched in copper, boron, and lead. This discovery was confirmed
and extended by Müller and Gentner (1973), who also found enrichments of zinc,
chlorine, and bromine. The latter authors compare the enrichment to that
observed in the orange soil of Apollo 17. The enrichments in chlorine and
bromine are by factors of around 20; for copper and boron the factor is more
like 5.
PETROGENETIC THEORIES
Volatilization
Cohen (1960b, c) deduced from the Ga/Ge ratio in tektites as compared with terrestrial glasses, that tektites had suffered severe differential volatilization. His measurements were questioned by Taylor and Sachs (1960) and Schnetzler and Pinson (1963). Taylor (1961) measured the distillation of alkali elements during ablation by comparing flanges and cores of australites; he found losses of alkali elements of about 20% for sodium and 5-10% for the other alkalis. Greenland and Lovering (1962, 1963) studied the relation of tektite compositions to those of granites and shales; they found that tektite compositions were derivable from granites, but not from shales. Taylor and Kolbe (1965) found little difference, even in volatile oxides other than CO2 and water, between Henbury subgraywacke and the impact glass derived from it.
In a series of papers, Walter and co-workers (Walter, 1967; Walter and Adams, 1967; Walter and Giutronich, 1967; Walter and Shadid, 1970) have put forward the suggestion that the sequence of tektite compositions represents progressive volatilization, starting from a composition like that of the more silicic Muong Nong tektites (82% silica). The key point in these papers is the discovery that, in an oxygen-rich atmosphere, silica is a relatively volatile oxide, exceeded in a volatility only by potash.
Walter’s suggestion was criticized by Chapman and Scheiber (1969). They referred to the above-mentioned results of Taylor and Kolbe (1965). They further argued that surface volatilization would not change the bulk composition, while volume ebullition (boiling) would produce a pumice, which is not observed. Diffusion in the available time is much too slow. Chapman and Scheiber further noticed that some Muong Nong tektites could not ever have been strongly heated (they have irregular voids, which would have become round or ellipsoidal if melting had occurred) yet are near the low-silica and of the normal tektite sequence. They also found that copper volatilizes so readily that 90% will be lost before 1% of the total mass. It follows that tektites which contain copper in normal amounts cannot have suffered severe losses of total material. Finally, the trend of the relation of magnesium and calcium to aluminum is at right angles to the trend to be expected for differential volatilization.
It is clear from Figs. 28-33 that differential volatilization has played a role in the history of tektite material at some time. The issue is between (1) Walter’s idea that the loss of volatiles occurred at a very late stage, during a violent heating event occurring in an oxidizing atmosphere such as the earth’s; and (2) an early volatilization, giving rise to the possibility that tektite composition can be used to make inferences about conditions in the region where they were formed (e.g. the deep interior of the moon).
The evidence at present seems to be against the first hypothesis.
Magmatic differentiation versus sedimentary differentiation
Chapman and Scheiber (1969) considered the possibility that the major element composition of tektites may result from magmatic differentiation under conditions of total pressure which are less than those for parallel terrestrial rocks. They note that the alkali basalts seem to come from deeper in the earth than the tholeiitic basalts; they regard some tektite compositions as a natural extension of this distinction. In particular, they suggest that the higher silica content of tektites (as compared with terrestrial acid igneous rocks) and their lower content of alkali elements and other elements with large ionic radii is the result of magmatic differentiation at low pressure. (They are thinking of the lunar interior, where the maximum pressure, at the center, is 47 kbars.)
Chapman and Scheiber reject a sedimentary origin for
tektites on the ground first pointed out by Lowman (1962) and later by Chao
(1963) and Tatlock (1965), that tektites show a much narrower field of
composition then sedimentary rocks; and any given tektite family shows much
less compositional range than a group of sandstones.
Tektites and achondrites
Tektites were compared with achondrites by Cassidy (1958) who noted that, as compared with generally similar rocks, both tektites and achondrites tended to be more silicic. Kvasha and Gorshkov (1961) made the same point, adding that both tektites and achondrites are relatively deficient in alkalis. Morgan (1969) noted that the correlation line between uranium and thorium in basaltic achondrites would approach the figure for tektites.
A direct connection of tektites with the parent body of the
achondrites does not seem plausible at present; but it is important to remember
that the basaltic achondrites resemble lunar basalts in their chemical
composition. The affinities noted may actually be telling us something about
processes of magmatic evolution on a body of low gravity and low water content.
GASES IN TEKTITES
Beck (1910) cited work by Brun to the effect that when tektites are heated, the principal gases given off were CO2 and CO in roughly equal amounts, H2, SO2, and traces of N2. Döring and Stutzer (1928) found similar proportions; both analyses were very different from those for obsidians.
H. E. Suess (1951) agreed generally with Brun (as cited by Beck) on the gas content of the rock; it is 0.1-0.3 cm³/g; Suess found chiefly CO, with some CO2 and H2; he also found considerable amounts of water, especially in an australite. The carbon found corresponds to about 50-150 ppm carbon in the tektite, and is thus of the order of the values given by Muenow et al. (1971) and Dolgov et al. (1969a, b) for organic compounds in tektites.
In the vesicles, Suess (1951) found less than 10-3 atmosphere. O’Keefe et al. (1962) identified Ne, He, O, and O2 in a large vesicle of a bediasite using an electrodeless discharge. The neon and helium appeared to be at about the same level as in the atmosphere, and had probably diffused in. Zähringer (1963b) confirmed the finding of neon and helium in nearly empty vesicles. In other vesicles, Zähringer found N2 and argon in their atmospheric ratio to one another. The work was extended by Müller and Gentner (1970) and by Jessburger and Gentner (1972). These workers found N2, Ar, Kr, and Xe in the same relative proportions as in the atmosphere, and with the same isotopic abundances as in the atmosphere. They do not feel that these vesicles have been filled by leakage from the atmosphere, because the CO2 is a major component, and O2 is greatly impoverished as compared with the atmosphere. On the other hand, we may note that in the soil, the air (“soil air”) is depleted in O2 and enriched in CO2. Since the tektites had been above ground for a considerable time before they were studied, and since they were outgassed in vacuum for several days before they were crushed, it is necessary to suppose either that the leaks were very slow, or that they had actually become stopped up in the course of time.
Jessburger and Gentner also find CO; this, they feel, rules
out soil air. On the other hand, it might have been a true initial constituent
of the gases, as reported by the early workers. The gaseous mix which
Jessburger and Gentner report, with CO2 greatly in excess of CO, and
with minor quantities of free oxygen, would not be in equilibrium with tektite
glass. It is hard to believe that it was ever in contact with tektite glass at
high temperatures.
COMPARISONS OF COMPOSITIONS WITH IMPACT MATERIALS
Ries crater glass versus moldavites
Cohen (1961) suggested that the moldavites originate from the Ries Kessel, a large impact crater in South Germany. On the basis of chemical comparisons, Chao (1963), Von Engelhardt and Hörz (1965), Preuss and Sassenscheidt (1966), Von Engelhardt (1967), and Barnes (1969) have all concluded that the moldavites cannot be derived from Ries impact glass, including certain small dense pieces of unusual homogeneity. A chemical comparison is given by Chao (1963); Table VIII from Von Engelhardt (1967) compares a suevite (Ries impact glass) with moldavite composition.
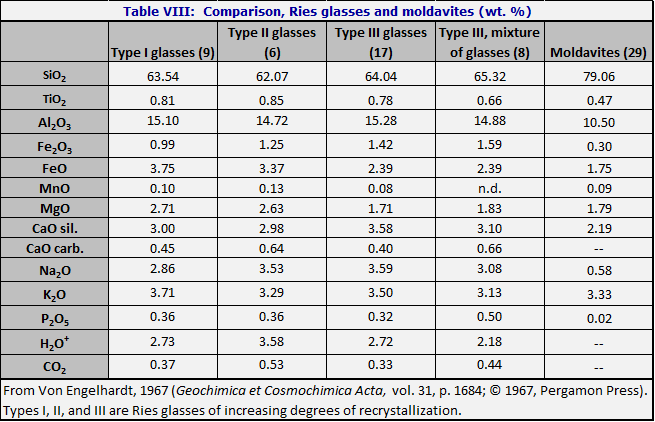
A number of investigators have suggested that although the
moldavites cannot be connected chemically with the impact glasses, which in
turn are related to basement rocks, they may be related to mixes of Mesozoic
sediments (Preuss and Sassenscheidt, 1966; Preuss, 1967; Bouška
et al., 1973). Preuss had difficulty explaining the comparative abundance of
CaO in this way. Bouška et al. further explored the trace element composition
of the local claystones and sandstones, without success. A convincing source
material for the moldavites has not been found.
Bosumtwi crater glass versus Ivory Coast tektites
Cohen (1963) suggested that the Ivory Coast tektites are the product of an impact at the Bosumtwi crater, in Ghana, at 6° 32’N latitude, 1° 23’ W longitude (O’Connell, 1965). From the chemical standpoint the best evidence is as shown in Table IX. Clearly the white glass listed in Table IX has nothing to do with the Ivory Coast tektites. The Student t-test has been applied to the green, gray and black glasses; the probability of no better agreement is shown in the last column of the table.
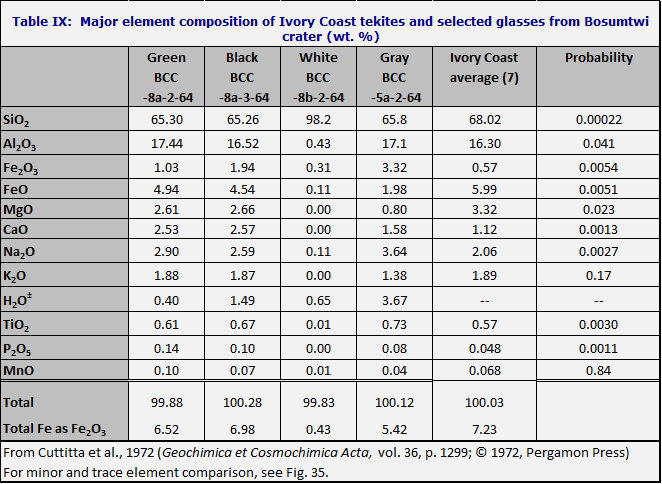
If the differences were due to accidental errors of measurement, or to random variations from sample to sample, we would expect that the calculated probabilities of no better agreement would range uniformly from 0 to 1. For instance, out of 30 trials, we would expect that the probability of no better agreement would it turn out to be less than 0.033 (1/30) once; less than 0.10 three times, and so on. A distribution of this kind is in fact observed when the Henbury subgraywacke is compared with the Henbury impact glass. But for the comparison of Bosumtwi glass with the Ivory Coast tektites, the distribution is entirely different; the probability values cluster around zero. It follows that the Ivory Coast tektites cannot be regarded as related to the Bosumtwi class in the same way that the Henbury glass is related to Henbury subgraywacke; i.e. if the Ivory Coast tektites are from Bosumtwi then we have not identified the source material.
There is, however, a remarkable chemical resemblance between the two, as shown in Fig. 36. In particular, Ivory Coast tektites are different from other tektites in the rare earth pattern; the heavy rare earths are slightly lower relative to most tektites. The Bosumtwi class is like the Ivory Coast tektites in rare earth pattern (Schnetzler et al., 1967). The tektite Th/U ratio is 2.9, instead of the 5 or 6 generally found in tektites; and the Bosumtwi rock (not specifically the glass) is similar (Rybach and Adams, 1969a, b).
The ferric/ferrous ratio in the Bosumtwi glass is 0.2 and 0.4 for the green and black glasses respectively; for the white and gray Bosumtwi glasses it is 2.8 and 1.7; for the tektites it is usually less than 0.1 and in all cases less than 0.15. The water content of the Bosumtwi glasses is 0.4-3.7%; for the tektites it was not included, yet the mean sum is 99.96% (incorrectly given as 100.03%) which indicates an unmeasurable water content.
No chemical analyses of the Bosumtwi rocks have been reported which resemble the bottle-green microtektites of the Ivory Coast strewn field.
In conclusion it appears that
the Bosumtwi white and gray glasses have no apparent relation to the Ivory
Coast tektites. The black and green
glasses show some striking resemblances to Ivory Coast tektites, but there are
also serious discrepancies. It is clear that the two rocks are not chemically
identical, but the degree of chemical similarity is surprising, and much closer
than in the case of the Ries and the moldavites.
Aouelloul crater glass versus local (Zli) sandstone
Monod (1952), on the basis of analyses by Campbell-Smith and Hey (1952a, b), believed that the Aouelloul crater glass was chemically so different from the local Ordovician sandstones (Oujeft, and overlying Zli) that the glass could not come from the sandstone. Chao et al. (1966b) analyzed specimens of Zli which bore a much closer resemblance to Aouelloul glass. Discrepancies remained, however, not only in ferric/ferrous ratio and water content, but in total iron and in CaO. Chao et al. suggested that the iron discrepancy might be due to an impacting iron meteorite. They noted, without giving data, that there were also serious discrepancies in the trace elements Ni, Co, Cr, V, Cu, and Sc. C. S. Annell has kindly permitted publication (Table X) of some new, systematic trace element analyses of the Zli sandstone specimens loaned by R. Fudali from a suite chosen to give a good sampling of the Zli. Nickel was around 10 ppm in the sandstone, but was at the level of 330-440 ppm in the glass.
O’Keefe and Annell (unpublished, 1974) noted that the application of the Student t-test indicated highly significant differences between the sandstone and the glass in the major elements. A 2% iron deficiency in the sandstone compared to the glass should call for at least 1000 ppm nickel if the extra iron is due to an impacting meteorite; the actual amount is 215-430 (Table X).
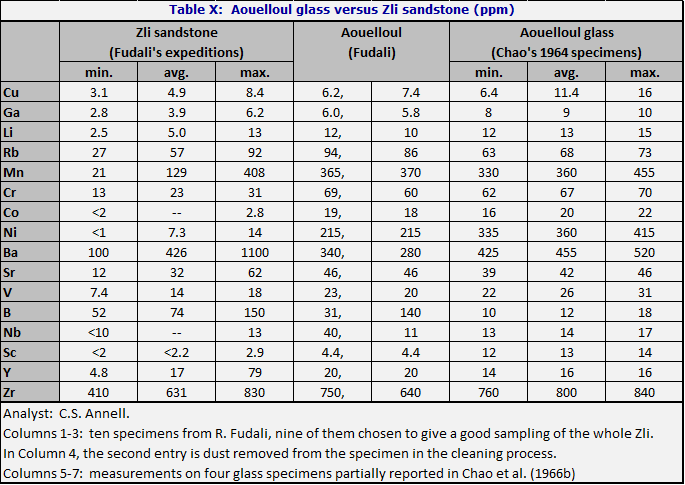
Here as at Bosumtwi it is clear that investigations up to this point have not turned up any rock which could be the source of the Aouelloul glass, in the sense that the Henbury subgraywacke is the source of Henbury glass. The failure is much more significant than at Bosumtwi, because the crater is only some 300 m in diameter, versus 10 km at Bosumtwi; the volume of rock disturbed is perhaps 40,000 times less. The strata at Aouelloul are horizontal, and the Zli is at the top – in fact it is eroded away over most of the surrounding area.
COMPARISONS WITH LUNAR ROCKS
12013 compared with the high-magnesium tektites
O’Keefe (1970), Mason and Melson (1970, p. 115) and Chapman (1971) have drawn attention to the resemblance of lunar sample 12013 to certain high-magnesium tektites. The resemblance in the major elements is striking; however, when the minor and trace elements are considered, the discordances are large, particularly in barium, chromium, yttrium, zirconium (Chapman, 1971), and also the rare earth elements, hafnium, thorium, and uranium (Showalter et al., 1971).
Rock 12013 is inhomogeneous; there is a dark portion, which resembles the lunar rock called KREEP, and a lighter more acidic portion. Chapman (1971) draws attention to the fact that the compositional trends observed in 12013 parallel those observed among the high-magnesium tektites (see Figs. 37-39). It may be significant that in both cases the compositional trends suggest mixing rather than a liquid line of descent.
Glass (1972b) finds that the bottle-green microtektites (which presumably continue the high-magnesium series toward lower silica content) have Cr2O3 up to about 0.2%, implying chromium up to 0.14%; this bridges most of the gap between 12013 and the high-magnesium tektites. Other trace element data on the bottle-green microtektites is not available.
Lunar soil particles
Studies of lunar soil particles (Reid et al., 1972a, b; Glass et al., 1972) showed that a component is present in the glass fraction at the level of about 2-5 particles per thousand of a type which is often called granitic. Much of the material is more potassic than any tektite (Table XI), but some will bear comparison with a high-calcium tektites. There are very few analyses of this material.
It should be kept in mind that all known tektites have survived on earth for a period of at least several hundred thousand years. It is known that the durability of glass decreases with increasing content of potassium; caution is called for in drawing inferences from the lack of potassium-rich tektites.
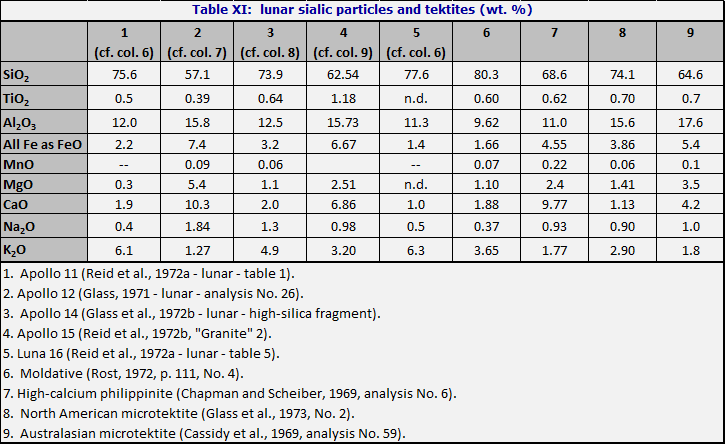
Libyan Desert glass and lunar tridymite and cristobalite
Libyan Desert glass has a superficial chemical resemblance to a sandstone of the very common type called quartz arenite (Pettijohn et al., 1972) and earlier called orthoquartzite. Quartz arenite is defined as having 95% quartz or more. However, as can be seen from Table XII, the relative proportions of oxides other than silica are strikingly different. For the quartz arenites they tend to resemble the relative abundances found in acid igneous rocks, or else to consist largely of the iron oxide which often cements the sand grains.
For Libyan Desert glass, on the other hand, TiO2 is almost half as abundant as Al2O3; CaO is about ten times as high as MgO; and Na2O ten times as high as K2O.
D. Futrell has pointed out in an unpublished note kindly communicated that the same relations are observed in lunar tridymites (see Table XII). The proceedings of the Lunar Science Conferences have numerous analyses of lunar tridymites and cristobalites; it is found that the relations shown in Table XII are very common, although not universal.
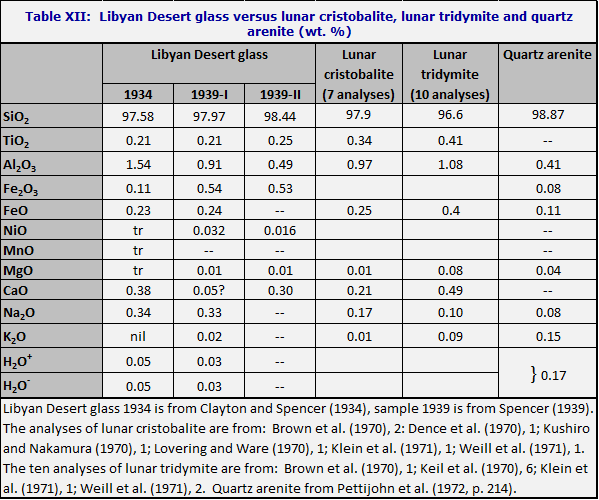
BOTTLE-GREEN MICROTEKTITES AND MESOSIDERITES
The bottle-green microtektites resemble to some extent the silicate portions of mesosiderites (meteorites which are mixtures of stone and metal, with the stone predominating). Duke and Silver (1967) suggested that the silicate portion of the mesosiderite Estherville might be a model for the parent material of two other meteorite types, namely eucrites and howardites. Since eucrites resemble lunar mare rocks, and howardites resemble (chemically only) certain green lunar glass spherules, it is of special interest to compare the silicate portions of Estherville with bottle-green microtektites. The comparison in Table XIII is with the average Ivory Coast bottle-green microtektite.
Note the higher Al2O3 content of the bottle-green microtektites. The significance of the relation noted here is not that bottle-green microtektites and mesosiderites come from the same place but rather that the two rocks both represent (imperfectly) some kind of primitive composition such as might be expected in the deep interior of a planet.
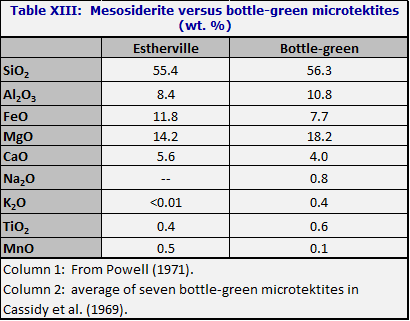
SUMMARY
Among terrestrial rocks, the intermediate igneous rocks and the sandstones resemble tektites most. Sandstones have a slight advantage, because they can combine a high silica content with enrichment of the mafic components. Tektites differ systematically from terrestrial rocks in their low water content, low ferric/ferrous ratio, and low abundances of the volatile elements; in these respects tektites resemble lunar rocks.
Specific chemical comparisons of tektites with him tektites from terrestrial meteorite craters are not encouraging except in the case of the comparison between the Bosumtwi crater in Ghana and the Ivory Coast tektites; here, although chemical identity does not exist, there is a remarkably close resemblance in several respects.
Few comparisons have been made between tektites and lunar rocks. There are resemblances in major elements between lunar specimen 12013 and the high-magnesium tektites; between some lunar soil particles and some microtektites; and between Libyan Desert glass and lunar tridymite and cristobalite. Trace element analyses are available only for the comparison of 12013 with the javanites; the resemblance is not close.
It appears that the most interesting elements for many of these comparisons are the neglected volatile elements on the right side of the periodic table; it is hoped that these can be studied in the future.